Invention for In vivo Live 3D Printing of Regenerative Bone Healing Scaffolds for Rapid Fracture Healing
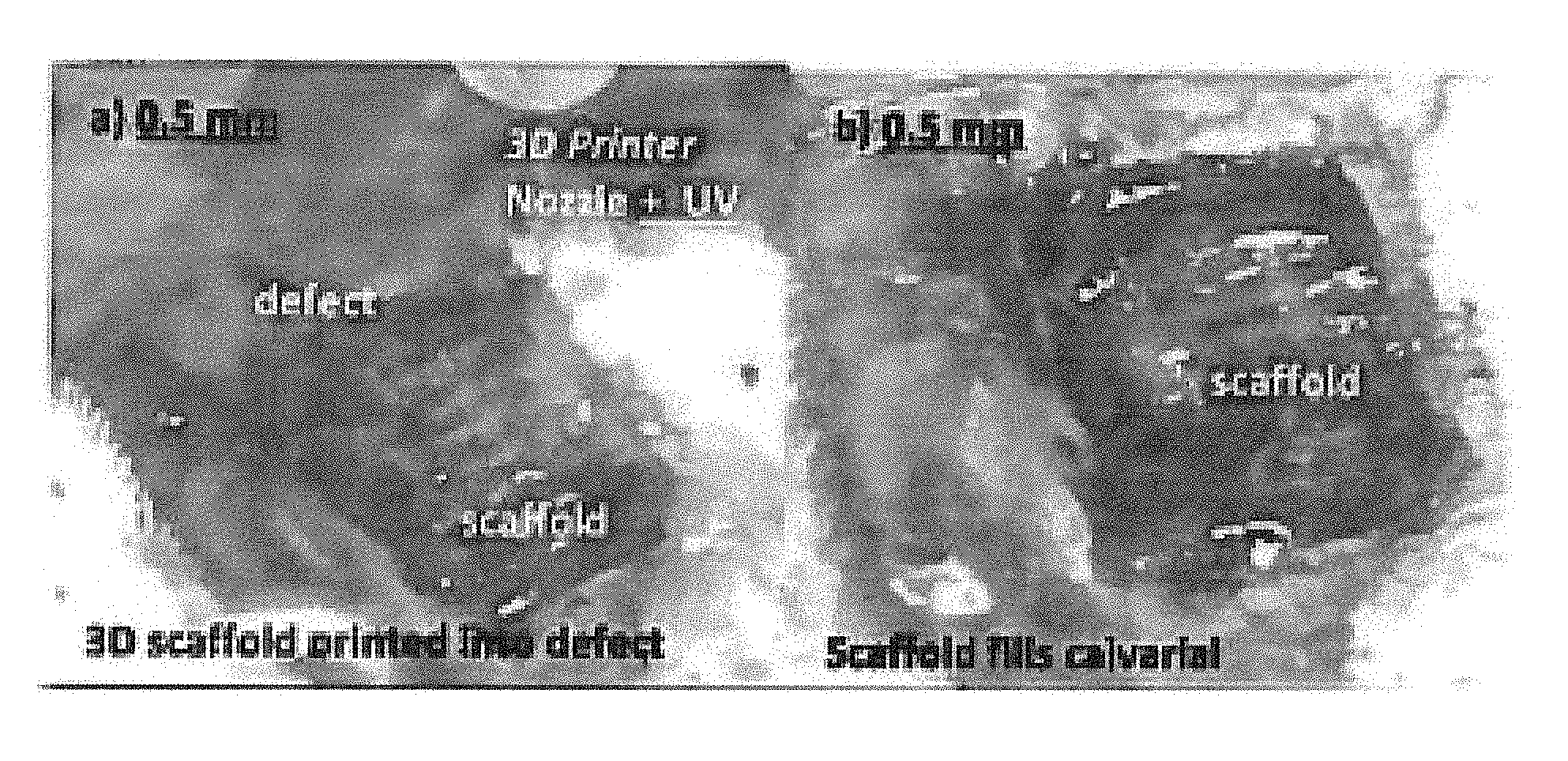
Invented by Venu G. Varanasi, Azhar Ilyas, Philip Roger Kramer, Taha Azimaie, Pranesh B. Aswath, Tugba Cebe, Texas A&M University System, University of Texas System
Fractures are a common occurrence, with millions of people worldwide experiencing bone injuries each year. Traditional methods of fracture healing, such as the use of casts or metal implants, have limitations in terms of their effectiveness and the time required for complete recovery. In vivo live 3D printing of regenerative bone healing scaffolds, on the other hand, provides a revolutionary solution that addresses these challenges.
This cutting-edge technology involves the use of biocompatible materials, such as hydrogels or bioceramics, that can be 3D printed directly onto the fracture site. The scaffold acts as a temporary support structure, promoting the growth of new bone tissue and facilitating the healing process. The ability to customize the scaffold’s shape and structure according to the patient’s specific needs is a significant advantage of this approach.
One of the key drivers of the market for in vivo live 3D printing of regenerative bone healing scaffolds is the growing demand for faster fracture healing solutions. Patients and healthcare providers are increasingly seeking alternatives to traditional methods that can significantly reduce the healing time and improve overall outcomes. In vivo live 3D printing offers the potential to achieve these goals by providing a more efficient and targeted approach to bone regeneration.
Moreover, the use of 3D printing technology allows for the creation of complex structures with precise control over porosity and mechanical properties. This level of customization is crucial for successful bone healing, as it enables the scaffold to mimic the natural bone structure and facilitate the integration of new tissue. As a result, patients can experience improved functionality and reduced risk of complications.
The market for in vivo live 3D printing of regenerative bone healing scaffolds is also driven by the advancements in additive manufacturing technologies. The development of bioprinting techniques and the availability of biocompatible materials have significantly contributed to the feasibility and scalability of this approach. As the technology continues to evolve, it is expected that the cost-effectiveness and accessibility of in vivo live 3D printing will further improve, making it a viable option for a broader range of patients.
In conclusion, the market for in vivo live 3D printing of regenerative bone healing scaffolds for rapid fracture healing is witnessing significant growth due to the increasing demand for efficient and personalized bone regeneration solutions. This innovative approach offers the potential to revolutionize the field of orthopedics by providing faster healing times, improved functionality, and reduced complications. As technology continues to advance, it is expected that in vivo live 3D printing will become a standard practice in fracture treatment, benefiting millions of patients worldwide.
The Texas A&M University System, University of Texas System invention works as follows
Bio-Inks, and compositions containing bio-Inks, are described. The bio-inks can also be used to repair or regenerate 3-D tissues by forming biodegradable scaffolds at a specific tissue site. Specific methylacrylated chitosan and methylacrylated gel hydrogels are disclosed, formulated with a silicate component (such a laponite) and/or a chemical or photo initiator, and/or a crosslinking agent. These preparations can be used in kits for tissue repair at the point of care. “We also demonstrate superior, more complete tissue regeneration (up to 99.85% within 4 weeks when applied in situ), rapid in situ bone formation and tissue repair.
Background for In vivo Live 3D Printing of Regenerative Bone Healing Scaffolds for Rapid Fracture Healing
Nearly 4,000,000 procedures of bone grafting are performed worldwide every year, at a cost estimated to be 2.3 billion dollars. This is expected to increase to 3.4 billion dollars by 2023 [30]. Around 600,000.000 of these are done in the US.
Bone grafts can be used to replace large amounts of bone lost due to fractures, trauma or any other disorder that may affect bone structure and function. These types of bone loss can lead to critical size defects, which are usually non-unions without grafting. Bone grafts should have sufficient mechanical properties to support the formation of new bone in the defect, and they must be osteogenic and absorbable to maintain continuity during bone regeneration. CSD is currently treated with allografts, autografts, and sometimes xenografts. These materials, however, can cause disease transmission and donor site morbidity. They can also trigger acute immune responses, which can lead to bone rejection and graft resorption [33]. Resources for autografts or allografts are also limited. There is an urgent need for new bone replacements that can quickly heal these defects in order to reduce the discomfort of patients and medical costs.
The treatment must be given as soon as possible after the fracture has occurred and should facilitate the turnover of bone in 4-8 weeks.
The current approach to bone repair has been described as involving the fabrication of scaffolds using 3-D printing technology. The fabricated scaffold can be implanted in a patient to repair a small or large bone fracture. The conventional methods used to fabricate 3D scaffolds require a lot of time and effort, but also do not allow for precise control over their architecture. Rapid prototyping is a technique which can be classified into additive and subtractive methods. The popularity of additive rapid prototyping is due to its ability to create more complex shapes and hollow structure. ARP is used more and more for tissue engineering [38] as well as craniofacial reconstructive surgery [37]. APR is currently used in preoperative treatment planning for craniofacial reconstructive surgery by creating a 3D model of the surgical site and performing the surgery using the model.
ARP is a broad category that includes many different methods including stereolithography, fused deposition modeling, direct metal laser sintering, laminated object manufacturing, electron beam melting, selective laser sintering, laser engineered net shaping, and 3-dimensional printing (3DP). 3DP does not require heat for its functionality which makes it useful for cell or growth factor incorporation. This feature made 3DP an attractive method for tissue engineering [39]. Robocasting or direct ink writing (DIW) is a subcategory of 3DP that is based on a computer aided fabrication method that uses extrusion of the ?ink? while moving in all three axes to make a 2D layer. By adding these 2D layers on top of each other, a 3D object can be created. The robocaster allows precise control of micro patterning by determining the dimensions of filaments, the size and shape of pores and the percentage of porosity of the scaffold [40-42].
There are only a few biomaterials which can be 3D-printed and implanted into the body, despite extensive research [42]. Bioceramics, such as TCP and HA, as well as biopolymers, such as PLA, PGA and PLGA, are among these materials.
The use of fixative materials or resorbable polymers has been described in the past as a way to repair bone fractures. Fixative metals and resorbable polymer material used in bone repair have several disadvantages. These include, among others, the lack of bioactivity. The patient’s healing time is prolonged and often incomplete. Metal implants are not reabsorbed into the body and remain fixed in the bone of the patient. The patient’s bone is not replaced in a way that allows for optimal replacement.
As noted, biopolymers are also used to repair bone fractures. The biopolymers currently used to heal bone fractures lack the strength needed to support rapid turnover of bone, among other disadvantages.
Existing 3-D printing techniques have also been found to be inadequate for the repair and replacement of fractured bones, primarily due to the fact that they require a significant amount of lead time before a scaffold can be constructed. The 3-D methods described previously do not offer an alternative to pre-fabricated scaffold implants for bone repair or a biocompatible, visco-elastic, material that is suitable for bone cells integration and bone growth.
Craniofacial reconstructive surgeries pose a unique challenge in terms of bone repair due to the complexity of this area and its proximity with vital tissues. In order to perform these surgeries, a custom-made implant had to be made prior. US PUB 20150054195 describes, for instance, pre-fabrication methods for bone grafts. Fabrication of a custom implant is time consuming, and creates a delay in when a patient may undergo a corrective/reconstructive surgery. The risk of a surgery not being successful or delivering results that are less than acceptable to the patient increases when surgical intervention is delayed. “A new surgical technique is required to treat bone defect with the shortest possible delay without sacrificing precision in the repair or implantation of the bone.
The medical arts still need alternative and improved techniques and materials for bone fracture repair. These materials and methods must be strong enough to provide immediate treatment of the fracture without a scaffold and have a better complete boney replacement than metal implants. Promote not adapted to use in direct printing materials into defects for immediate repair of the fracture/defect sites.
The present invention provides, in an overall and general sense, materials and methods to repair bone fractures, defects, and other bone deficiencies.
Method for 3-D printing in Vivo to repair tissue and bone defects:
In one aspect, the system is described for printing porous scaffolds in vivo. In certain embodiments, the process involves preparing a biosilicate slurry containing osteoinductive biopolymers and biosilicate microparticles; and applying this slurry to a fracture site using a printer attached to a motorized duct and driven by a computer-aided design program.
The method of providing a regenerative scaffold is described. It comprises: selecting an optimal scaffold slurry, calibrating the printer’s parameters, recording the surface profileometry of the defect in three dimensions; programming the defect into the CAD software; engaging the head of the printer; filling the defective with the printed scaffold whilst maintaining a small space; and closing the skin and periosteum.
As part of the method for some embodiments, the bio-Ink will be fed in its final form into a delivery system, such as the nozzle on an automated 3-D printer, and applied as a series layers to the tissue site, whether it is an animal or a human.
In another embodiment, the method can include the step of preparing a bio-Ink first by combining a certain amount of lyophilized MAG with a specific quantity of nanoparticles based on silicates (such a hydrous sodium magnesium silicate (Laponite?) This bio-Ink will have a suitable viscosity that will allow it to be extruded through a nozzle having an extrusion component, such as a needle. This bio-Ink has a viscosity which allows it to be extruded from a nozzle with an extrusion element, such as needles. The needles or other extrusion components have a size between 14 and 32 gauge (in some embodiments a gauge 30 (0.2mm) dispenser tip).
The “printing” (i.e. delivery) of bio-Ink to a tissue site can be achieved by delivering a predetermined quantity of bio-Ink through a small gauge dispensing tip, such as an needle with a gauge of between about 14 (1.55mm) and 32 (about 0.10 mm), into or around a defined site, sufficient to cover specific dimensions within about 0.01mm to about 1 mm (or within 0.1mm to 0.5mm) of the perimeter of the target tissue area. The direct 3-D reconstruction techniques and materials will result in successful bone regeneration within less than 4 weeks for a bone defect. This includes 80% of the target tissue area, or between 85-90%, or 86%-99.9833%.
The conditions in which the bioInk is delivered will be strictly regulated to ensure that filaments of bio-Ink are formed within the tissue. The temperature and rate of deposition, for example, shall be controlled to prevent any compromise to the extruded bioInk material or any compromise to the filaments which may be formed during cross-linking the bioInk material. (Cross-linking must be initiated with a wavelength and intensity of UV light that is physiologically compatible and a cross-linking compound such as IRGA-CURE). Extrusion conditions and/or micropatterning processes are controlled to reduce and/or eliminate any possibility of tissue degradation or destruction at the site of treatment and around it. As filaments of material solidify or ‘cure,’ the conditions of deposition are optimized to create the best conditions for the formation of an appropriate biodegradable structure (having defined pore size between 300 m and 700m). The cross-linking agent is applied to the wound area, after exposure to UV light of a wavelength and intensity compatible with the human body (such as 365 nm at an intensity up to 50 mW/sq.cm) in the presence of the wavelength.
The method can provide different geometries and configurations for bio-Ink to be deposited at a desired location, including a single layer or multiple layers stacked or unstacked, a mesh configuration, triangular or rectangular configuration, or any other configuration that is best suited to the tissue site being repaired, for example.
Biocompatible Regenerative Bone slurry material:
Click here to view the patent on Google Patents.